On November 8, 2012, at a Stated Meeting held in Cambridge, Frank Wilczek, the Herman Feshbach Professor of Physics at the Massachusetts Institute of Technology, discussed the modern concept of substance and the nature of the Higgs particle. His presentation and the introduction given by Jerome Friedman, Institute Professor and Professor of Physics Emeritus at the Massachusetts Institute of Technology, follow.
Frank Wilczek is one of the most prominent theoretical physicists in the world. His theoretical work is characterized by its inventiveness, depth, and breadth.
He received his undergraduate education at the University of Chicago and did his graduate research at Princeton under the supervision of David Gross. He taught at Princeton University; the University of California, Santa Barbara; and the Institute for Advanced Study. In 2000 he was appointed as the Herman Feshbach Professor of Physics at MIT.
Frank has a number of significant achievements to his credit. But he is best known for his pioneering work in developing the theory of the strong interaction, known as quantum chromodynamics, or GQD for short. The strong force is the force that holds quarks together to form other particles, such as protons and neutrons; it also holds neutrons and protons together to form the atomic nucleus.
When I was a graduate student at the University of Chicago in the early 1950s, it was said that it would take a hundred years to understand the strong force. At the time there was a highly successful quantum theory of electromagnetism based on field theory. But field theory did not appear to be suitable for the strong interaction. However, in 1973 Frank, David Gross, and, independently, David Politzer discovered a field theory that successfully describes the strong interaction.
QCD predicts that the interaction between quarks will be weaker at short distances and get stronger at long distances. The first behavior is called asymptotic freedom. The latter feature is called infrared slavery, and it explains why individual quarks have not been found in nature, even though they have been seen inside the proton and neutron with the equivalent of a very powerful electron microscope.
QCD has been verified in numerous experiments over a wide range of energies. It is one of the pillars of the standard model, which is our highly successful theoretical description of the fundamental particles and their interaction. The standard model is considered one of the crowning achievements of twentieth-century physics.
For this pioneering work Frank was awarded the Nobel Prize in 2004, along with David Gross and David Politzer. In addition to the Nobel Prize, Frank has won many other honors. These include the Sakurai Prize, the Dirac Medal, the Lorentz Medal, the King Faisal Prize, the Lilienfeld Prize of the American Physical Society, and the Particle Physics Prize of the European Physical Society.
Frank’s research has covered a broad range of topics in condensed matter physics, astrophysics, and particle physics. In addition to his work on GQD, he predicted the existence of a new particle in nature called the axion. This particle is a plausible candidate for dark matter, and various laboratories are searching for it.
Frank also invented a new form of quantum statistics that has important implications in condensed matter physics, and he has made a number of contributions to understanding deep issues in the implementation of QCD in particle physics and astrophysics.
His talents go well beyond his scholarly work. He has written a number of highly successful books that bring modern physics to the public. The latest of these is titled The Lightness of Being. He is currently finishing a novel. In addition, he plays the piano and the accordion and performs in rock bands.
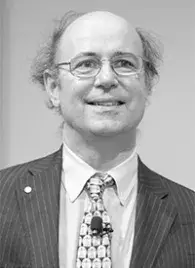
We learned in the twentieth century that matter is a very different thing than everyday experience suggests. To understand it deeply, we need entirely new concepts. These concepts have brought a new level of beauty and coherence to fundamental physics, a foundation of our world-picture. They also invite new questions and ambitions.
The modern understanding of matter has many aspects. I won’t be able to do justice to all of them in the brief time available. Instead, I am going to focus on two big ideas. The first is that all matter is like light. The second is that space is a material.
Until recently people thought there was a basic dichotomy between matter and light. It seems obvious — so obvious, that it went unquestioned for centuries. But it is not so.
Many relics of outmoded ideas are embedded in everyday language, and so here. Since I — and modern physics — want to consider light and matter as one thing, in what follows I will use “substance” to denote that thing. I will use the word “matter” as it is traditionally used, to denote the kind of thing that makes rocks and people. In the literature of modern physics, “matter” is usually taken to include light, but for present purposes we will have to step back from that usage.
As many of you know, in quantum theory, a product of the twentieth century, some of the main distinctions between light and matter, as understood previously in physics, went away. A great achievement of nineteenth-century physics was to understand that light consists of wave-like disturbances in electric and magnetic fields. The fields, as dynamical entities, fill all space. Matter, on the other hand, was thought to be built up from tiny particles that occupy discrete bits of space. We learned in the twentieth century, with the development of quantum theory, that this distinction is superficial.
If you want to understand the behavior of matter on small scales — within its constituent atoms, say — then matter must be described, as light is, using space-filling fields. But they are not precisely the same kind of fields that we had before; they retain a vestige of the old particle concept. An example will be helpful here. We can visualize how an electron settles down when it is bound to a proton in a hydrogen atom. In any of its orbitals, called stationary states, we can picture the probability of finding an electron in different places. That field of probabilities is what the electron’s wave function encodes. We can use brighter areas to show where the probabilities are large, but in principle nonzero probabilities extend out to spatial infinity, in any of the states. These visualizations, which make quite attractive patterns, are not fantasies. (They should seem fantastic, but they are not fantasies.) We use programs like Mathematica and Photoshop to create the images, but the description of hydrogen they incarnate is based on equations that have been verified with extraordinary accuracy — in important respects, to parts in trillions. There can be little doubt that this is the way nature works, and that the images are an honest portrayal of profound understanding.
Conversely, light can sometimes behave as if it were made of particles, called photons. I’ll say more about that later. In the more accurate, unified treatment both matter and light obey the same kinds of equations. Perhaps you have seen a classic paradoxical image, that when apprehended from one point of view seems to be a rabbit but from another point of view seems to be a duck. In reality the image is neither, or both: It is what it is, a dabbit. Similarly, we have wavicles.
The wave/particle synthesis transcends one major contrast between light and matter. But there are several other apparent differences between the usual conceptions of light and matter that need to be explained, if we are going to get a unified concept.
For example: Light gets created and destroyed easily, whereas matter is permanent; it gets rearranged but not created or destroyed. Light travels at the speed of light, and is always on the move, whereas material particles, such as electrons and protons, travel at less than the speed of light, and can come to rest. Light transfers energy from one place to another. Matter, when it moves at all, transfers mass from one place to another. And perhaps what is most striking, light is in a deep sense a single thing, whereas matter seems to be made out of several different things. Atoms of the different chemical elements are made of electrons and different kinds of nuclei; the nuclei are made out of protons and neutrons; the neutrons and protons are made out of up and down quarks and gluons. The list of matter’s building blocks gets more sophisticated, but it doesn’t converge to a list with just one entry.
Physics today is justifiably ambitious. We can realistically aspire to a unified picture that gets down to just one thing. So, among the rest, we want to eliminate the dichotomy between light and matter. The tale is not finished, but one by one those apparent distinctions between light and matter have fallen away. Recently we have completed a major new chapter of the story, as I’ll now explain.
Light moves at the speed of light. In the context of special relativity, this means that the particles of light, photons, have zero mass. And that is a really nice feature. When you study the equations of physics, you find that particles with zero mass satisfy the equations that are the most beautiful, the most conceptually tight, the most consistent, and the most elegant.
So, to bring unity and beauty to our description of nature, we would like to make the world from building blocks, fundamental particles, that have zero mass. Unfortunately, many of the fundamental particles we know do not cooperate. Electrons, for instance, do not move at the speed of light in empty space, but always slower.
What can we do about that? When physicists do not like how the world works, we have learned there is an interesting fallback position: We imagine a better world, and then see if we can somehow gracefully mess that world up, to get ours.
The new development is that we have found a good solution to the mass quandary. It involves an idea that was theorized almost fifty years ago, but has only now come to experimental fruition. The resolution, following the fallback strategy I just mentioned, is to imagine that space is filled with a material, a “cosmic molasses,” that slows some things down (including electrons and quarks, but not photons). If you remove that material, imaginatively, particles such as electrons and quarks will move at the speed of light, and they will be described by the beautiful equations of massless particles. The observed fact that electrons and quarks don’t move at the speed of light will reflect the evil influence of that all-pervasive cosmic molasses, rather than an intrinsic shortcoming.
The molasses metaphor is kind of icky. Here is a nicer metaphor: Imagine that a race of intelligent fish evolves in the oceans of a water-rich planet. Those fish get so intelligent that they start to think about physics and the laws of motion. They carefully study how things move, and they derive some very complicated laws, because the way things move through water is very complicated. The push-back of the water itself complicates motion.
For a long time, our fish struggle with their equations, until one day a fish genius, Fish Newton, figures out another way to describe things. You can get by with much simpler laws of motion, she proposes, by removing water from your equations. The fish of course had always lived surrounded by that stuff we call water, and had come to take it for granted, not realizing they could remove it, imaginatively. Once you do that, Fish Newton demonstrates, you can start with simpler laws–Newton’s laws of motion. Then you put the water back in, you recover the complicated laws that fish physicists had derived before, which describe everyday motion as observed in their world.
The big news is that we are like those fish. We live in a universal, cosmic ocean. We can get a simpler, more beautiful description of the world, more elegant laws of motion, more elegant laws for all of physics, by postulating that a material fills the whole world everywhere and slows things down and gives various particles mass.
It’s an old idea, as I mentioned, and we have been using it for decades to get more beautiful equations, which have been quite successful. But there has been a big problem looming over it. We learned a lot about matter in the twentieth century. Through studies ranging from intricate chemistry to systematic, precise, violent experiments with accelerators, we have developed a rich, full account of how the physical world works. We made a tremendous amount of progress. We made an inventory of the forces, and discovered two new ones (the strong and weak forces, supplementing gravity and electromagnetism). We discovered many new fundamental particles and understood their interactions. It would be hard to overstate the power and accuracy of our understanding of substance. But in all of our explorations, nothing we discovered had the right properties to make the cosmic ocean. The charm quark doesn’t do it, nor the beauty quark, nor the top quark, nor photons nor gluons. None of those particles is up to the job.
As our beautiful equations using the cosmic ocean idea went from triumph to triumph, our confidence in the existence of that medium grew. Since no known material would provide it, physicists postulated a new one: the Higgs field.
Fortunately, that bold hypothesis has observable consequences.
In the quantum description of the world the minimal units, or quanta, of any field appear as particles. For instance, photons are the quanta of the electromagnetic field. Light appears continuous when you have lots of it. But if you have very dim light, you find that it breaks up into little packets, each with the same finite energy (for monochromatic light). Those are the quanta, photons, the minimal units, of light. Gravitons are the quanta of gravity. They have not yet been detected, and for the foreseeable future they probably won’t be, because they interact too feebly. But theoretically we are sure they are there. Gluons are the quanta of the nuclear force fields. They were first inferred theoretically, and then found experimentally in one of the great triumphs of twentieth-century physics.
The Higgs field also has quanta, called Higgs particles. They are the minimal units of the world-filling material substance we postulated, to legitimize the equations of our dreams.
Since many of the properties of the Higgs particles could be predicted in advance of their observation, we could infer what they had to look like. And so we could recognize them when we saw them. The great news of July 4, 2012, is that thanks to a massive international effort at CERN’s Large Hadron Collider, now we have.
The LHC is a giant twenty-six-mile circular tunnel. Inside it, we have protons moving at within one part in a million of the speed of light. Two beams of those very energetic protons circulate in opposite directions around the tunnel. Once you have gone through the trouble of accelerating the protons to such high energy you want to keep them, so you have them move in a circle. You have to use powerful superconducting magnets — lots of them — to coax the protons. The reason the ring has to be so big, and the magnets so powerful, is simply that when protons move so fast it is extremely hard to deflect them from straight-line motion. The magnets’ power requirements would be exorbitant, if they were ordinary magnets. So they have to be superconducting magnets, and this requires that you keep them at a very low temperature, two degrees Kelvin. And that requires a lot of specialized plumbing, using liquid helium. If any of that plumbing goes wrong, you can have catastrophic failures. Getting all this to work involves truly heroic engineering.
The intellectual point is to explore our theories of the fundamental way the world works, using those protons. That step presents lots of challenges, too. I’ll describe one especially interesting and important one. When two protons smash together, what actually collides, usually, are two gluons, one from each proton. If your goal is to make Higgs particles, that is problematic. For the Higgs particle, in line with its role as the quantum of molasses, couples to particles proportionally to their mass. (Remember, the mass arises from that coupling.) But gluons, like photons, have zero mass. So gluons don’t couple to the Higgs particle directly at all.
Fortunately, though, gluons do manage to couple to Higgs particles indirectly, in a remarkably beautiful way.
Gluons couple directly to all kinds of quarks, including the very heavy (and very unstable) top quarks and top antiquarks. A top quark–top antiquark pair can come to be and then pass away after a short time, spontaneously. That possibility is a special case of quantum uncertainty, or if you like quantum fluctuations. We call such evanescent pairs “virtual particles.” The top quark–top antiquark pair, being very heavy particles, are very happy to couple to the Higgs particle. And so the gluons get to couple to the Higgs particle indirectly, with the help of deep quantum physics, through those intermediate virtual particles.
Once you produce the Higgs particle, it occasionally decays into two photons. Since photons, like gluons, have zero mass, that decay process also must go through intermediate virtual particles. That circumstance raises a remarkable possibility. Besides the particles we know about, other very heavy particles, that we have not yet observed, could also be contributing, in their virtual form. And so it is especially important to measure the properties of the Higgs particle accurately, because they open a new window into the unknown.
The collisions that produce Higgs particles are a small subset of all the collisions that occur at the LHC, and even within that subset the Higgs particle is one particle among dozens of the particles emitted. So you have to understand and measure things extremely well in order to pick out this particular process from all the processes we already understand and don’t regard as so interesting anymore. Or, to use a different metaphor, if you are looking for a needle in a haystack, it is really important to know exactly what hay looks like. Physicists have a saying, “Yesterday’s sensation is today’s calibration.” Astonishing things we were proud of discovering in the late part of the twentieth century are now taken for granted and used as stepping stones, to reach higher.
It is one thing for a theorist to draw doodles showing what the process will look like. Actually detecting those photons is quite another. The cost of the LHC was approximately five billion euros. The whole project is our civilization’s answer to the pyramids of Egypt. In this case, it is a monument to human curiosity and determination.
The detectors are especially fantastic. The Atlas detector would fill a fair-size aircraft hangar. It is stuffed with state-of-the-art, even beyond-state-of-the-art, electronics designed to reconstruct what happens in those violent collisions, or “Little Bangs.”
You can’t resolve the tiny time scales over which all the action takes place by taking snapshots. It is more like studying the ashes, after an explosion, in order to reconstruct what the explosion itself was like. Lots of particles, typically several dozens, emerge. You want to see how many there are, which ones they are, and how fast and in which directions they are moving. Essentially all of them are moving very close to the speed of light, but maybe one is moving within one one-hundred-thousandth of the speed of light, while another is moving within one one-millionth of the speed of light. Those tiny differences carry crucial information. You have to be very fast and accurate about measuring particle velocities and distinguishing the different kinds of particles.
Thanks to this grand endeavor, now we have firmly established the existence of a material that fills the universe and changes the properties of things we know about. That answer suggests some wonderful questions.
If space is — or, more flexibly, is filled with — a material, could that material change with time? Specifically, could it have melted or boiled away early in the Big Bang, when things were very hot? And could it be differently organized elsewhere?
In fact, it has already proved fruitful to think that the properties of space-filling material — or, you might say, of space itself — change with time. The early universe could have been very different in its fundamental properties. In particular, it could have had a sort of negative gravity, which triggered a process of very rapid inflation. That might explain quite a bit about the universe we see. Cosmic inflation is a topic for another talk, but it is important to mention how our ideas hang together.
We are also licensed to speculate, since the properties of space are negotiable, that things could be very different elsewhere. This leads to a concept called the multiverse, the idea that if you got far enough away you would find that the effective description of the world is quite different from what we have here. The world might, for instance, look as if it had a different number of dimensions. Or instead of one kind of electromagnetism, you might have two with different kinds of photons and different versions of electric charge simultaneously. Or you might even find a universe in which there is no QCD.
Could all the apparent distinctions among elementary particles, not just their masses, arise from their differing reactions to cosmic fields? That possibility would allow for a truly unified description where all the particles, all the fundamental building blocks of nature have equivalent properties, but are made to look different due to complications introduced by world-filling substances. Detailed investigation shows this idea is not only viable, but explanatory, and productive of testable consequences. In this way Einstein’s dream of unification has evolved from mystic faith to quantitative science.
The details of that story are a movie for another time, still in production, but I can show you the trailer. The movie, which is actually a sort of sequel to “The Modern Concept of Substance,” is called Desperately Seeking SUSY.
SUSY is short for supersymmetry. It is the next great target for discovery at the LHC. SUSY involves the idea that space has another set of dimensions that is very small and that we don’t see (yet). In fact, the new dimensions are not dimensions of space in the ordinary sense; they are something quite new: quantum dimensions. The central innovation is that to describe positions in superspace, instead of ordinary numbers that satisfy x × y = y × x, you have to use a new kind of numbers, Grassmann numbers, for which x × y = - y × x. That strange minus sign makes a big difference. The quantum dimensions are not just small, but in a sense discrete. You can think of them as just having two sheets. You can explore a quantum dimension fully in one step, because a second step leads you to nowhere: x2 =0.
If you step into the other sheet of the quantum dimension, you don’t arrive in a different place, in the usual sense; rather, you turn into a different kind of particle. So, if you were an electron, you would turn into something called a selectron, which retains some of the electron’s properties, such as the same electric charge, but has much larger mass and is a boson instead of a fermion. Roughly speaking: If you were a force-mediating particle, you will become a material particle, and vice versa. That is the essence of supersymmetry, physically. Thus SUSY would transcend an annoying dichotomy in our current descriptions of nature: that is, the distinction between matter particles and force particles, or in the jargon, between fermions and bosons. That dichotomy, you will appreciate, is a modern, sophisticated descendant of the old divide between matter and light.
But the reason I don’t merely admire SUSY, but love her, is more concrete. If you assume that the new super-particles exist, and you consider their effects as virtual particles, you discover a marvelous quantitative consequence. For if you extrapolate the known laws of physics to short distances, making corrections for the effects of those additional virtual particles, then you find that the different interactions come to have equal strength. Thus unification of all the interactions we know, including gravity, will at last mature from a vague hope into a quantitative phenomenon.
I hear sirens singing. They sing that empty space is a medium that, given SUSY, reconciles perceived differences. And they sing another suggestive detail: The observed mass of the Higgs particle, it turns out, is nicely consistent with SUSY’s preferences. Like Odysseus, tied to the mast, listening to all this, I am entranced, hoping the sirens sing true. Over the next few years, through further experimental exploration, we will find out whether they have been teaching us or teasing us.
Some of you might know Caravaggio’s painting of St. Thomas inspecting the wounds of the Lord. I have always admired St. Thomas, and thought of him as the patron saint of experimental physics. He wants to see the actual wounds.
But the painting also includes the one whose wounds Thomas wants to examine, the patron saint of theoretical physics, who said, “Blessed are they who do not see and yet believe.”
© 2013 by Jerome Friedman and Frank Wilczek, respectively