on the future of global energy
Energy is the greatest concern of our future. The rising living standards of a growing world population will increase global energy consumption dramatically over the next half century. The challenge for science, and particularly for the discipline of chemistry, is to meet this energy need in a secure, sustainable, and environmentally responsible way. This essay will frame the magnitude of the problem, show the insufficiency of conventional energy sources to meet these needs, and pose an alternative solution.
By 2002, the global population burned energy at a rate of 13.5 TW. (One TW equals 1012 watts, or 1012 joules per second. This unit is convenient because it normalizes energy use per unit of time.) In the next forty-five years, this burn rate will rise with alarming alacrity. To gain a sense of the scope of the problem, we can perform a simple but powerful analysis: we can multiply a country’s TW consumption per person by the projected global population of 9 billion people for the year 2050 (see table 1). For example, if 9 billion people adopt the current standard of living for a U.S. resident (which takes 1.1361 × 10-8 TW of energy to sustain), the world will need an astronomical 102 TW of energy in 2050.
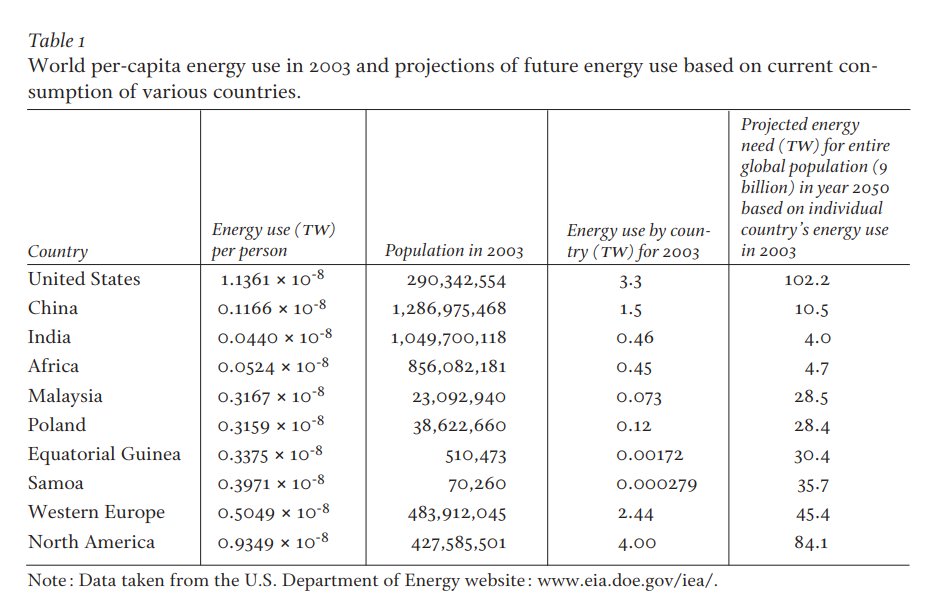
The next three entries–China, India, and Africa–are cause for concern. These countries–and, in one case, an entire continent–have very low per-capita energy use but possess the largest populations on our planet. Since energy consumption scales directly with a country’s GDP, global energy use will increase drastically as China, India, and Africa modernize. So how much energy will the world need in 2050? It depends. If everyone adopts Equatorial Guinea’s current living standards, we will need 30.4 TW by 2050. Or in the case of Samoa’s, we will need 35.7 TW. Both are well below what we will use if everyone in the world consumes energy at North America’s (84.1 TW) or Western Europe’s (45.4 TW) current rates. Conservative estimates of energy use place our global energy need at 28–35 TW in 2050.
Even with extreme conservation measures (maintaining a 102 TW standard of living with only 28–35 TW of energy available will require conservation measures that are far beyond the human experience), we will still need an additional 15–22 TW of energy over our current global base of 13.5 TW. If this sounds simple to achieve, then consider the total amounts of possible energy from the following sources (all figures come from the World Energy Assessment, http://www.undp.org/seed/eap/activities/wea/; a more comprehensive presentation of these numbers can be found on http://nsl.caltech.edu/energy.html):
- From biomass, 7–10 TW: This is the maximum amount of biomass energy available from the agricultural landmass of the planet, which excludes the area needed to house a population of 9 billion. Obtaining this quantity would require harvesting all crops exclusively for energy.
- From nuclear, 8 TW: Delivering this TW-value with nuclear energy would take the construction of eight thousand new nuclear-power plants. In other words, over the next forty-five years, we would have to construct one new nuclear-power plant every two days.
- From wind, 2.1 TW: We could only gather this amount of energy from wind by saturating all global landmass in class 3 and greater with windmills. (‘Class’ refers to an area’s wind-energy potential: a class-3 area has winds of 5.1 meters per second at 10 meters above the ground, the minimum necessary for sustainable energy generation.)
- From hydroelectric, 0.7–2.0 TW: We could achieve this supply of hydroelectric energy by placing dams in all remaining rivers on the earth.
These scenarios are meant to illustrate the scale of the energy problem that confronts our global community. They assume no new advances in science and technology, e.g., the design of new reactor cores or genetically engineered biomass. And in some cases, they are restrictive, e.g., most potential wind energy is over the ocean surface and not land. The point is that, even under the untenable circumstances outlined above, we can barely attain the necessary energy supply for 2050.
The message is clear. The additional energy we need by 2050, over the current 13.5 TW base, is simply not attainable from long-discussed sources–the global appetite for energy is simply too great. We could use more coal, oil, and gas. However, rising energy costs, energy security, and, perhaps most urgently, concerns for the environment, provide the drivers to take energy exploration from the oil fields to the laboratory bench. There, a carbon-neutral, renewable energy source must be discovered.
The principal environmental problem with the continued use of fossil fuels to supply the growing energy demand is the release of CO2 into the atmosphere. Atmospheric CO2 concentration during the last century has risen monotonically. Moreover, detailed analysis of the relative abundance of carbon isotopes confirms that this observed CO2 increase is the result of burning fossil fuels. The current CO2 concentration of 370 parts per million (ppm) is unparalleled in the last six hundred fifty thousand years, with CO2 levels ranging from 210–300 ppm until now. Unfortunately, atmospheric CO2 concentration will likely double, even triple, within the twenty-first century. While we cannot predict the consequences of this increase precisely, there is no question that we are perturbing the planet on an unprecedented scale. The effects of our actions on the earth are unarguably serious, but hopefully not catastrophic. It is thus imperative that the global community moves as quickly as possible to carbon-neutral energy sources.
Of the possible sustainable carbon-neutral energy sources, sunlight is preeminent. More solar energy strikes the Earth’s surface in one hour of each day than the energy used by all human activities in one year. If we could only mimic photosynthesis outside of the leaf–i.e., an artificial photosynthesis–then we could harness the sun’s energy as a fuel. Such a process would combine water and sunlight to produce hydrogen and oxygen. The hydrogen would then be combined with the oxygen in a fuel cell to give back water and energy. In the overall cycle, sunlight and water are converted to useful energy in the form of the fuels hydrogen and oxygen.
But there’s a catch. Using water and sunlight to make a clean, sustainable fuel to power the planet is a daunting endeavor, as we must uncover large expanses of fundamental molecular science in order to enable light-based energy-conversion schemes.
To emulate photosynthesis, we must be able to capture sunlight and relay it to catalysts that then act on water to rearrange its bonds and make the chemical fuel, hydrogen, and its by-product, oxygen. In designing these hydrogen- and oxygen-producing catalysts, we must take the following into consideration: The overall water-splitting reaction is a multielectron process, involving a total of four electrons. The development of a quantitative, predictive model describing single-electron reactions was a milestone achievement in chemistry in the last half-century. A similar understanding of multielectron reactions, however, has yet to be realized. Moreover, the transfer of four protons must accompany electron transfer–so we need to learn how to manage both electrons and protons. Finally, whereas chemists know how to catalytically rearrange energy-rich (i.e., reactive) bonds, we have yet to develop efficient bond-making/breaking reactions on energy-poor (i.e., stable) substrates such as water.
Scientists are currently working in each of those areas to advance the science of renewable energy at the molecular level. Some of the latest advances include discovering guidelines for the rational design of multielectron reactions and uncovering proton-coupled electron transfer (PCET) as a field of study at a mechanistic level. With the frameworks of multielectron chemistry and PCET in place, catalysts that can produce hydrogen and oxygen have been created. Though these are not yet ready for practical use, this will come in time with molecular reengineering. In any case, the development of these catalysts and the studies of their reactivity are revealing the principles needed to simulate photosynthesis. The creation of solar-produced fuels is only part, albeit a significant one, of developing a reliable solar-based technology. A U.S. Department of Energy report on a Solar Energy Utilization workshop (http://www.sc.doe.gov/bes/reports/files/seu_rpt.pdf) identifies a number of other basic-science needs: new photovoltaics to capture solar energy efficiently and relay it to the catalysts; new materials for safe storage of hydrogen and other fuels; the activation of other small molecules of energy consequence such as CO2; and an understanding of reactions of energy consequence at interfaces and at surfaces. Ultimately, the advancement of solar-energy technology depends on the implementation of basic-science discoveries, which require effective, responsible public-management and economic/social-science policies throughout the entire innovation cycle.
Clearly, the greatest crisis confronting us in the twenty-first century is the rapidly growing demand for energy. Because the chemical bond, and the manipulation of the energy within, lies at the heart of this endeavor, chemistry will likely play the most central role of all the sciences. What chemists do in the coming decades will determine whether or not we will bequeath to our planet the gift of the sun as its source of energy.